All published articles of this journal are available on ScienceDirect.
Electrospun Nanofibers for Tissue Engineering: Desired Properties
Abstract
Electrospun nanofibers have gained great attention in the biomedical industry, especially in tissue engineering, because of their interesting properties that promote cell growth and tissue cicatrization or regeneration, where any biological tissue can be beneficiated by choosing the proper biomaterials. Hence, the objective of this perspective article is to give an insight into the desired properties of the electrospun nanofibers dedicated to the tissue engineering approach. A high tensile strength, flexibility, reduced permeability of water, high surface area, biocompatibility, and biodegradability are some of the properties recognized and discussed to be more important for tissue engineering applications. The purpose of these properties is to mimic the surrounding tissue or create the optimal condition for the targeted cell growth. Despite all the reported literature, it still is missing a complete screening of the above mentioned properties specific to their respective target tissues.
1. INTRODUCTION
The value of the electrospinning technique has been reported over the last 10 years [1-13]. This technique leads to the production of versatile nanofibers that possess a diverse set of properties [3] and can be used in several applications such as tissue engineering [1, 2, 4, 12], drug delivery systems [4, 5, 7, 8, 10, 11], and biotechnology [6, 13], amongst others.
Among the interesting properties of electrospun nanofibers, the high tensile strength, flexibility, reduced permeability of water [14], high surface area [15], biocompatibility [2], and biodegradability [16], are a few mentioned.
The above properties can always be designed through the choice of a specific polymer [4] that is used as a matrix and can be functionalized with a great variety of biomaterials such as metals [9, 12], ceramics [1, 2] or other polymers [6, 13], these above biomaterials can be used to functionalize the nanofibers with extra properties.
Such is the case of the integration of an antimicrobial effect on the nanofibers thanks to some bioactive properties, for example, silver nanoparticles [12] and curcumin [13], or conductive properties by adding graphene [17], polyaniline [18] or having therapeutics effect loading pharmaceutical drugs in/on the surface of the nanofibers such as dexamethasone [10], sildenafil citrate [11], just to mention some examples.
Tissue engineering is one of the approaches beneficiated by the electrospun technology [1-4], because nanofibers create a tridimensional structure that simulates the extracellular matrix made by tissues (Fig. 1). These structures can be prepared with biomaterials that resemble the chemical composition of tissues, using natural biomaterials, for example, hydroxyapatite [1, 2] which promotes the regeneration of bone tissue. By the same time, the nanofibers are reabsorbed and become part of the tissue [19]. The main objective of tissue engineering is to avoid tissue and organ transplantation. Hence, natural and synthetic biomaterials are provided through the electrospinning technique of 3D tissue formation, which is regularly enhanced by the seeding of the cells into the material structure.
In Fig. (1), it can be observed that electrospun fibers with different diameters (yellow arrows) create a tridimensional scaffold that resembles the extracellular matrix of tissues.
Despite all tissues can be susceptible to the use of electrospun nanofibers for regeneration/cicatrization improvement, just a few of them have been extensively studied, such as bone [1, 2, 4, 14, 16, 17, 19], cartilage [20, 21], and skin [4, 12, 18], this can be due to the accessibility of the tissue and less complicated applicative experiments in universities or research institutes [7].
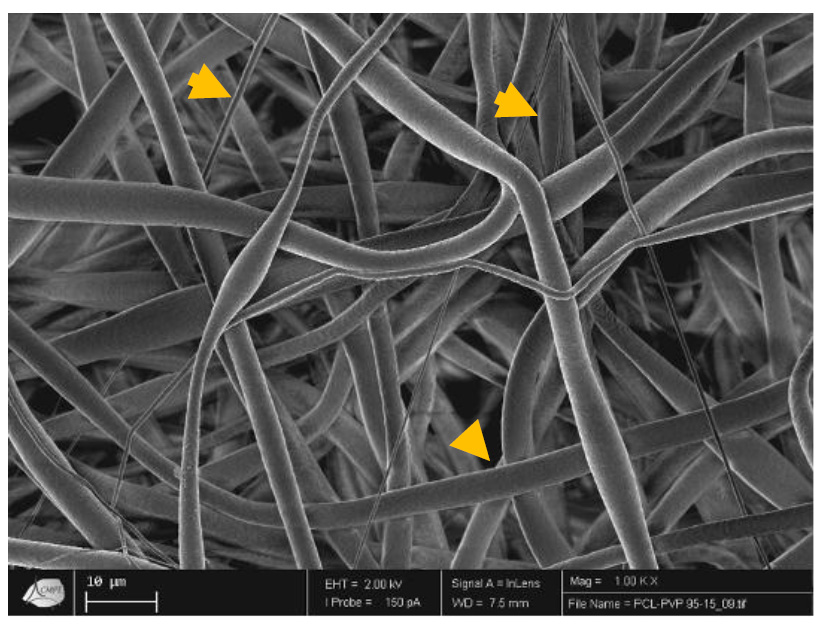
For tissue regeneration, bioactivity, biocompatibility, biodegradability, and adequate mechanical properties are the main factors to resolve in the design of an excellent system [12].
Bioactivity can be incorporated on the surface of the fibers in case the polymeric blend has no bioactivity by itself (such as collagen or hydroxyapatite [1, 2] whose sole presence increases the stimulation of bone formation), for example, antimicrobial agents [12, 13], proliferation enhancers such as growth factors [22], pharmaceutical drugs for tissue stress relief [23], being all these additions promoter of enhancers of the function of the 3D structure of the scaffolds.
Conversely, biodegradability offers a lifetime of the scaffolds to prevent overstaying its presence in the tissue and avoiding additional surgery or treatments, and this degradation time is dependent on the severity of the lesion and the rate at which the tissue is proliferating [24]. Moreover, degradation metabolites have to be carefully considered in designing an implantable scaffold because this bioproduct will lead to the success or failure of the membrane [25].
Mechanical properties of the scaffolds depend on the tissue, the zone of implantation, and handling requirements [12]. These properties vary and always are desired that the mechanical properties of the scaffolds assemble in the tissue. The adequate characteristics of the fibers are crucial to the successful use of the system due to their structural support for the newly formed tissue [26].
Finally, biocompatibility is the most important feature of the electrospun scaffolds for tissue engineering since the implanted materials have to realize a specific function with an adequate response from the surrounding tissue and not over stress [1, 2, 12]. With this property, cytotoxicity is avoided and tissue response is evaluated. The equilibrium between bioactivity, biodegradability, and mechanical properties is desired depending on the specific tissue and injury to treat [4, 22, 23].
Research groups that are dedicated to tissue engineering and proposed electrospun nanofibers need to carefully study all these features and demonstrate the feasibility of their systems. Biomedical systems always need not to be toxic and do not provoke chronic alteration of the tissue.
2. BIODEGRADABILITY
The biodegradability of the electrospun fibers depends on the chosen polymer used to fabricate the fibers. Biodegradable polymers propose significant advantages for disposable or fast consuming products in medical applications [27].
The poly (hydroxyalkanoates) (PHA), a class of naturally occurring poly (esters) that are secondary metabolites of microorganisms in excess of carbon source conditions, are among the most significant biodegradable polymers and are highly recommended for biomedical applications due to their biodegradability [27].
Moreover, biodegradable aliphatic polyesters (poly (lactide) (PLA), poly (glycolide) (PGA), and poly (lactide-co-glycolide) (PLGA) are the most characteristic synthetic polymers for tissue engineering and regenerative medicine. Despite that, aseptic inflammation is one of their disadvantages due to their acidic degradation creating conditions for further implantation [28].
The desired degradation time of the electrospun fibres varies depending on the application; some, like poly (vinyl pyrrolidone) (PVP) [29], are designed to disintegrate in seconds, while others, like poly (caprolactone) (PCL) [30], are intended to degrade over the years.
In Table 1 are enlisted some examples of biodegradable polymers that can be used in the electrospinning technique and have been used for tissue engineering.
Electrospun Fibers | Biodegradable Polymers | Degradation Time | Application | Refs. |
---|---|---|---|---|
PHBV | PHBV | 420 days | Osteoblast and fibroblast regeneration | [27] |
CTS/PLGA | PLGA | Several weeks to several months (55 days) | Amelioration inflammatory responses | [28] |
GT/PCL | PCL | 2 or 3 years | Postoperative Cardiac Adhesion | [30] |
PLCL | PLCL | 72 days at 50 °C, 177 days at 37 °C | Orthopedic shoulder surgery | [31] |
PPC | PPC | 8 months | Peripheral nerve regeneration | [32] |
CTS/PU | PU | 20-23 years | Soft tissue engineering | [33] |
PLA/PEG | PEG | 10-12 hrs | Endothelial cell regeneration | [34] |
3. MECHANICAL PROPERTIES
One of the main problems of the electrospun fibrous scaffolds is the lack of evaluation of the impact of solvent retention in fibers on the scaffold’s mechanical properties [35].
D'Amato et al. 2018, discussed that the retained solvent can act as a plasticizer, modifying the mechanical properties of the fibrous scaffolds such as brittleness and stiffness. In that sense, that study evaluated the retention of solvent and its effect in PGA, PLCL, and PET electrospun fibers through themogravimentric analyses. The obtained results showed that polymers that were electrospun below their glass transition temperature (Tg) held solvent and polymers that were electrospun above their Tg did not, which affect mechanical properties such as Young’s moduli, toughness and failure strain as the solvent evaporates [35, 36].
One of the most intriguing polymers utilised in the electrospinning process is poly (caprolactone), which has promising mechanical qualities, is less expensive than other polymers, is biocompatible, and has a slow rate of degradation, making it perfect for tissue engineering applications [36]. It is important to highlight that besides the polymers' properties, the fibers' thickness confer the mechanical properties. Hence, PCL possesses great mechanical properties for tissue engineering in trabecular bone, skin, blood vessels, liver, lungs, heart and kidney because its tensile module is about 62±26 MPa in PCL fibers of 440-1040 nm, which is higher than the tensile module of all the above tissues Table 2 [36].
4. BIOCOMPATIBILITY
Electrospun nanofibrous scaffolds possess morphological parallels with the extracellular matrix in tissues, which give them thanks to cell adhesion, proliferation, and cell function properties making them ideal for tissue engineering applications (Table 3) [44]. Biocompatibility is the most important feature in electrospun nanofibers intended for tissue engineering [1, 2] because the successful role of the electrospun fibers implanted in tissue is the main and final objective of any biomedical biodevices. It is important to remark that in order to achieve biocompatibility, the low cytotoxicity of the nanofibers is crucial [1-4].
Çakmakçı et al. 2012, combined UV curing and electrospinning technologies to produce methacrylatd cellulose acetate butyrate (CABIEM) electrospun nanofibers. The cytotoxicity of these nanofibers was evaluated using the MTT assay in human umbilical vein endothelial (ECV304) and mouse embryonic fibroblasts (3T3) cells. Also, a modified collagen presence in the CABIEM fibers was proposed in order to enhance cell adhesion and proliferation. Regarding their results, the CABIEM fibers appear to be non-toxic; authors discussed that cell viability was related to collagen proportion. The study encountered that cell adhesion and proliferation were improved as the collagen concentration increased [45].
In another study, conductive electrospun fibrous scaffolds of poly (pyrrole) (PPy) were proposed to rush the healing of damaged tissues. Authors tested different proportions of PPy in polymeric fibers and concluded that the presence of PPy enhanced conductivity. It is well known that conductivity in the electrospun fibers promotes cell adhesion, growth, and cell proliferation. Hence, conductive fibers can be proposed for tissue regeneration of the heart, nerve, skin, and other tissues where electrical signals are required for cell communication [46].
Electrospun Fibers | Cell Line | Biocompatibility Study | References |
---|---|---|---|
PCL/GP | Rat stem cells | Cell attachment and differentiation assay | [44] |
CABIEM | ECV304 and 3T3 cells | MTT assay | [45] |
PPy/CTS/COL | Fibroblast cells | MTT assay | [46] |
TPU/PDMS | Human skin fibroblast cells | MTT assay | [47] |
PHBV/PVA | HUVECs, SMCs and MSCs cells | Flow cytometry and immunocytochemistry | [48] |
Ginestra P. 2019, prepared different proportions of graphene loaded in poly (caprolactone)/cyclo pentanone electrospun fibers, presenting interesting mechanical behaviors. Statistically significant differences in mechanical and biological properties were related to graphene concentration. In the study, rat stem cells were exposed to these fibers that found a great relationship between the graphene presence, taking into account that graphene confers conductivity on the polymeric fibers. Also, a higher proportion of dopaminergic neurons were identified in the study related to higher percentages of graphene [44].
Finally, Drupitha et al. 2019, evaluated the biocompatibility of thermoplastic polyurethanes/ polydimethylsiloxane (TPU/PDMS) electrospun fibers using the MTT and cell proliferation assay with human skin fibroblast cells. It was found that fiber morphology, porosity, surface wettability, and biological and mechanical properties were influenced by the presence of the PDMS fraction [47].
CONCLUSION
Electrospun nanofibers have demonstrated their capacity to improve cell proliferation in different cell lines in vitro and in vivo. For the success of the fibrous scaffolds, it is necessary to test and design proper formulations to confer specific properties that will help the fibers interact with cells and the surrounding tissue. The high tensile strength, flexibility, reduced permeability of water, high surface area, biocompatibility, and biodegradability are the most interested properties for tissue engineering. However, not all the reported literature completely screen these parameters.
LIST OF ABBREVIATIONS
PHA | = Poly (Hydroxyalkanoates) |
PLA | = (poly lactide) |
PLGA | = (lactide-coglycolide) |
CONSENT FOR PUBLICATION
Not applicable.
FUNDING
None.
CONFLICT OF INTEREST
Luis Jesús Villarreal-Gómez is the Editorial Advisory Board of The Open Biomaterials Science Journal.
ACKNOWLEDGEMENTS
To Dr. Daniel Grande, East Paris Institute of Chemistry and Materials – CNRS, Paris, France, for taking the micrograph figure.